Mid-IR Dispersive Mirrors
Dispersive mirrors in the Mid-IR (1 – 6 μm) range can finally substantially aid the combination of various bulk materials, such as semiconductors (Si, Ge, GaAs) and dielectrics (fluorides, sapphire, YAG) as the straightforward means to compensate dispersion and compress ultrafast mid-IR laser pulses.
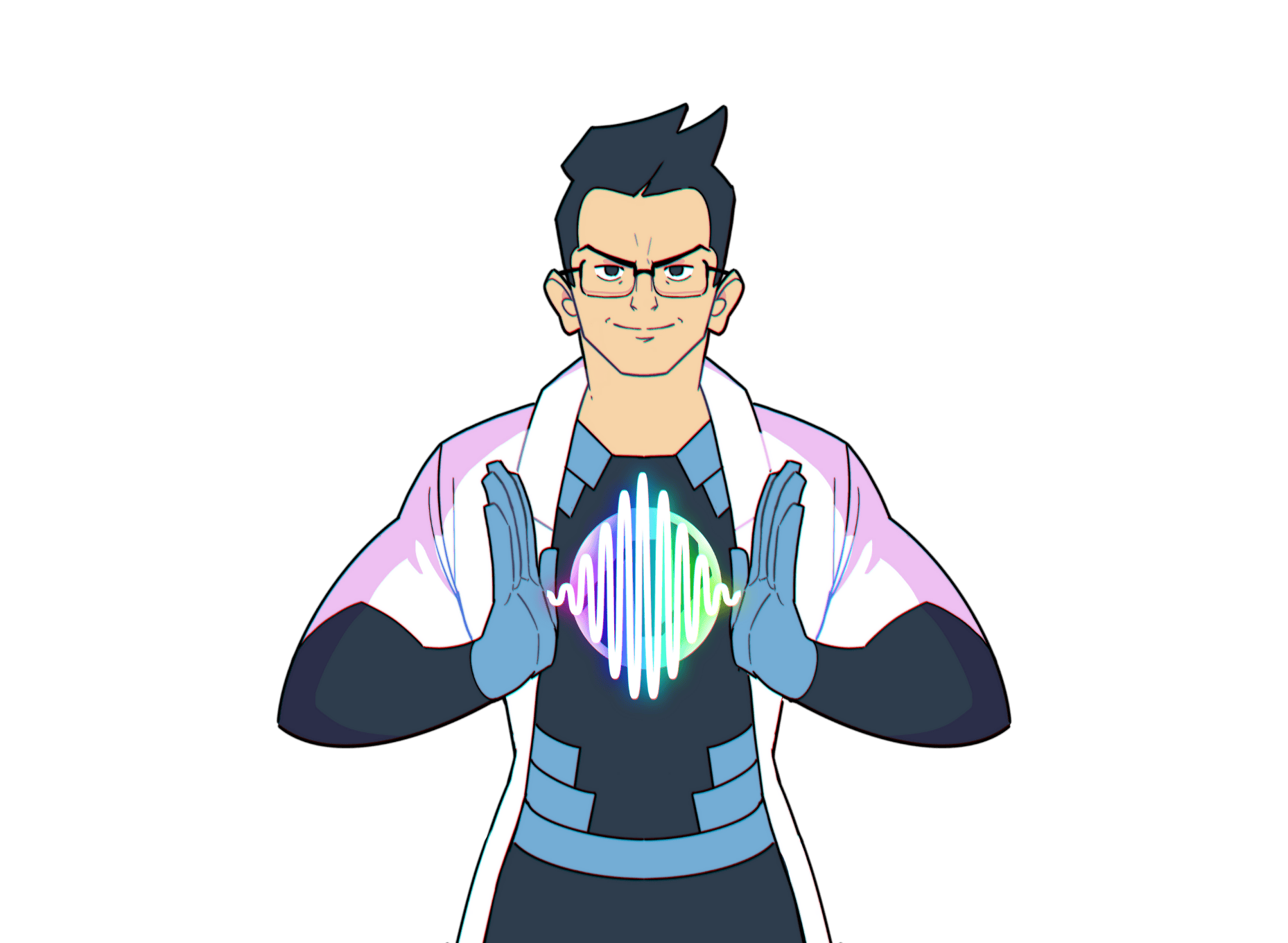
The problem
However, the availability of materials transparent in mid-IR spectral range is limited due to the presence of strong absorption bands. In addition, it can be difficult to find the right material combination to precisely compensate the complex phase characteristic acquired by the laser in the long optical path from the generation to the application.
Semiconductors and dielectrics have opposite signs of GVD, which opens a straightforward route to adjust the chirp. However, the specific TOD transferred to the pulse by material insertion is exclusively positive, which heavily limits the efficiency of recompression in the sub-3 cycle regime. Therefore, gaining control over the dispersion up to the 3rd order by specially designed dispersive optics plays a key role here.
Application field
Therefore, mid-infrared light sources are used for atomic physics experiments and HHG to investigate ultrafast processes at atomic time and nanometer spatial scales. HHG-sources are also mentioned as candidates for medical applications like coherent diffraction imaging in the water window. Such sources proved to be suitable also to quantify carrier envelope phase (CEP) variations of few cycle IR pulses on target.
Solution and benefits
Dispersive mirror
The produced mirror exhibits an average reflectance of R>98% over the 2150-3700 nm wavelength range and 0-25° angle of incidence for p polarization. As targeted in the problem description, the key feature of this mirror is its GDD value that is gradually increasing from -300 fs² at 2150nm to 300 fs² at 3750 nm. By using this mirror at two different angles (10° and 25°) GDD oscillations can be somewhat suppressed, leading to smoother spectral phase response. Theoretical reflectance and GDD curves of the mirror are depicted in Fig. 1.
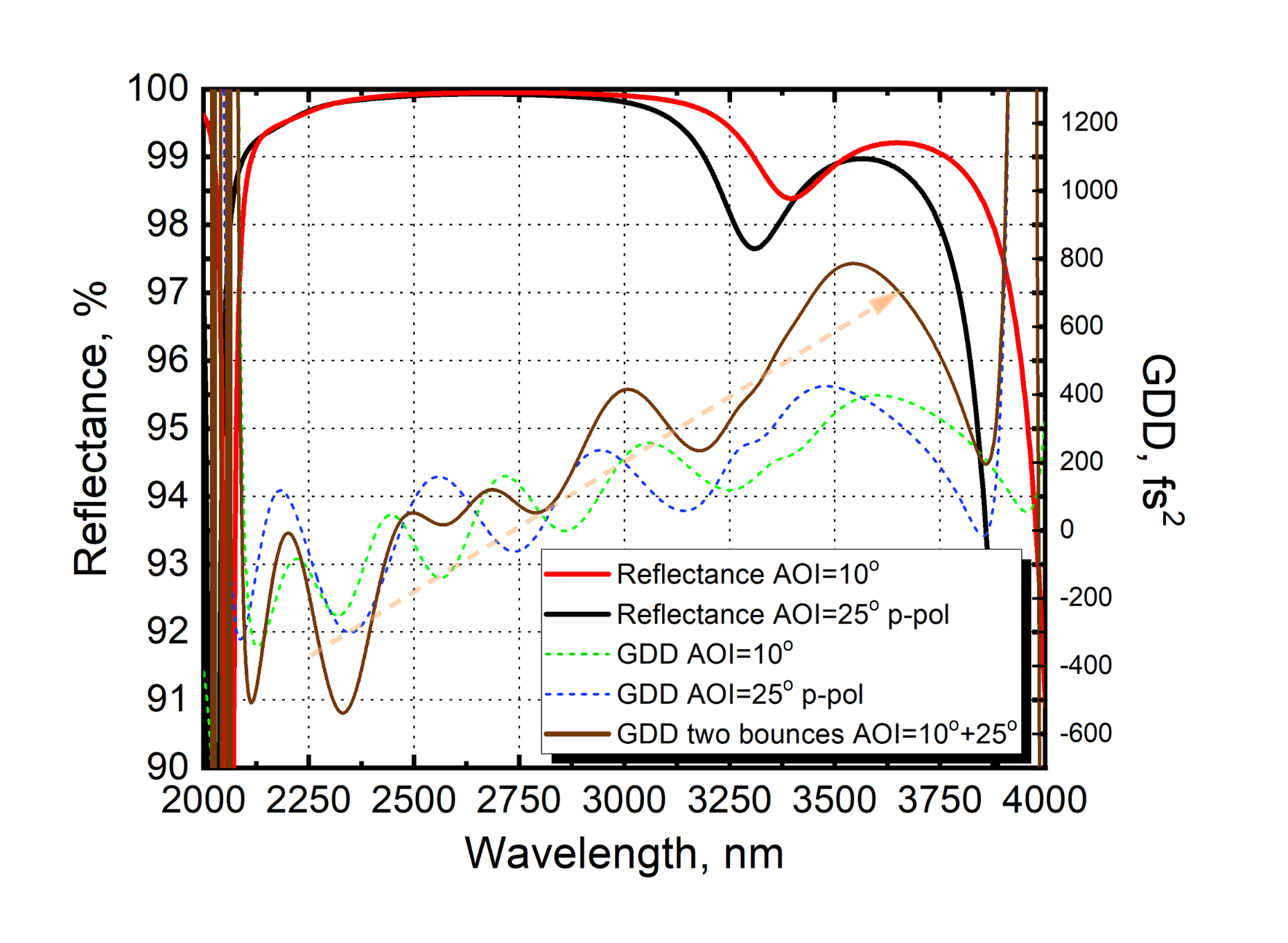
Since dispersive mirrors are extremely sensitive to manufacturing errors, characterisation of spectral phase shift was performed by a spectrally Resolved Interferometry (SRI) technique to quantify the actual GD performance of the mirrors and validate the designed phase curves. Mirrors were tested with a Mach-Zehnder interferometer setup in 2860-3480 nm wavelength range. The comparison between
theoretically calculated and measured group delay curves is shown in Fig. 2. The SRI measurements show good agreement between calculated and measured GD values at both 10° and 25° angles.
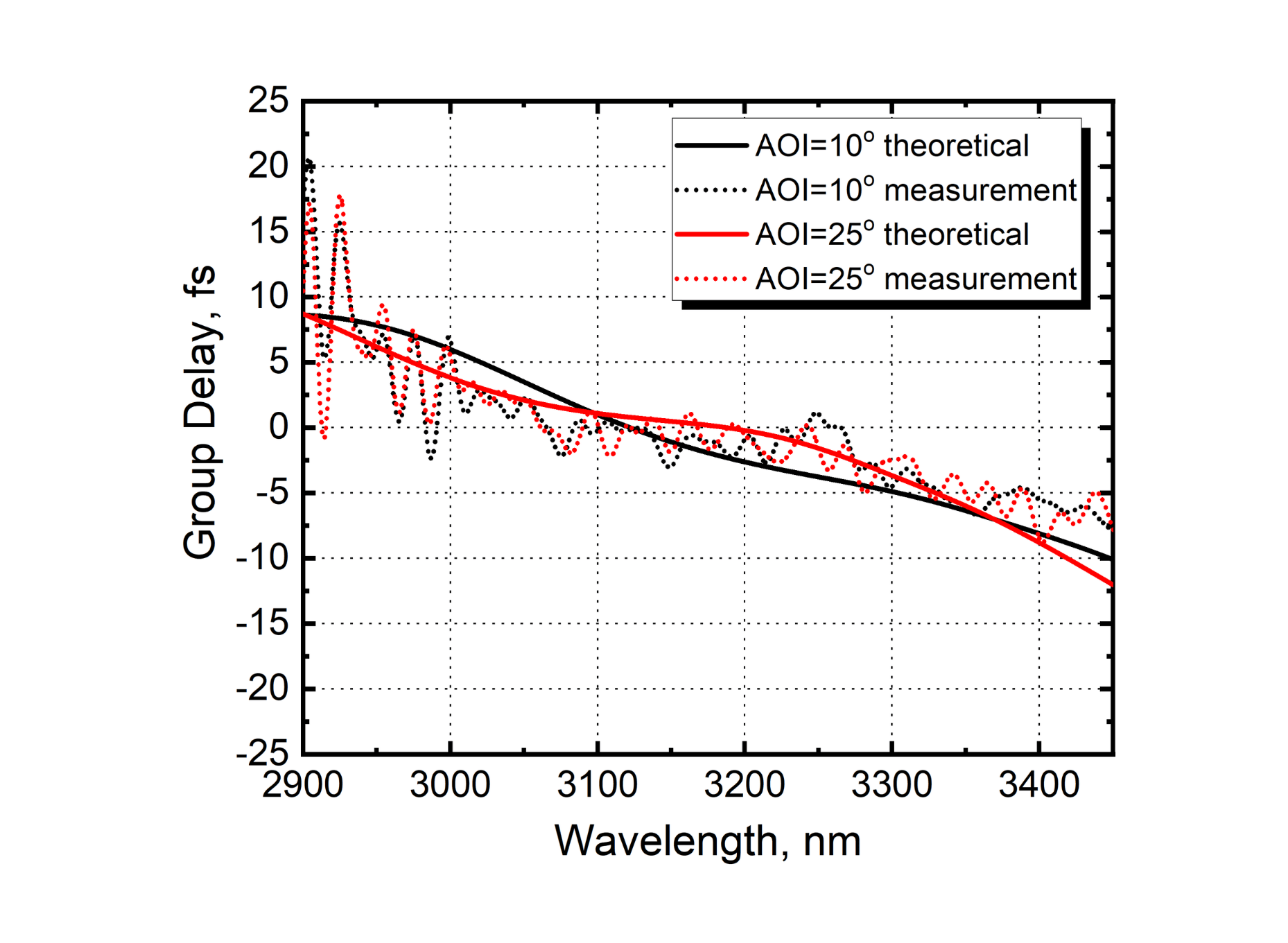
Finally, pulse compression efficiency of these mirrors was tested with spectrally broadened mid-IR pulses at ELI-ALPS. Laser pulses with 48 fs duration at 3100 nm central wavelength were focused into a spectral broadening unit consisting of BaF2 and Si windows positioned along the beam waist. The pulse spectrum after the broadening unit (Fig. 3) supports 18 fs Fourier transform limited (FTL) duration and has positive chirp. Recompression performed with the combination of bulk CaF2 and the dispersive mirrors, testing several configurations.
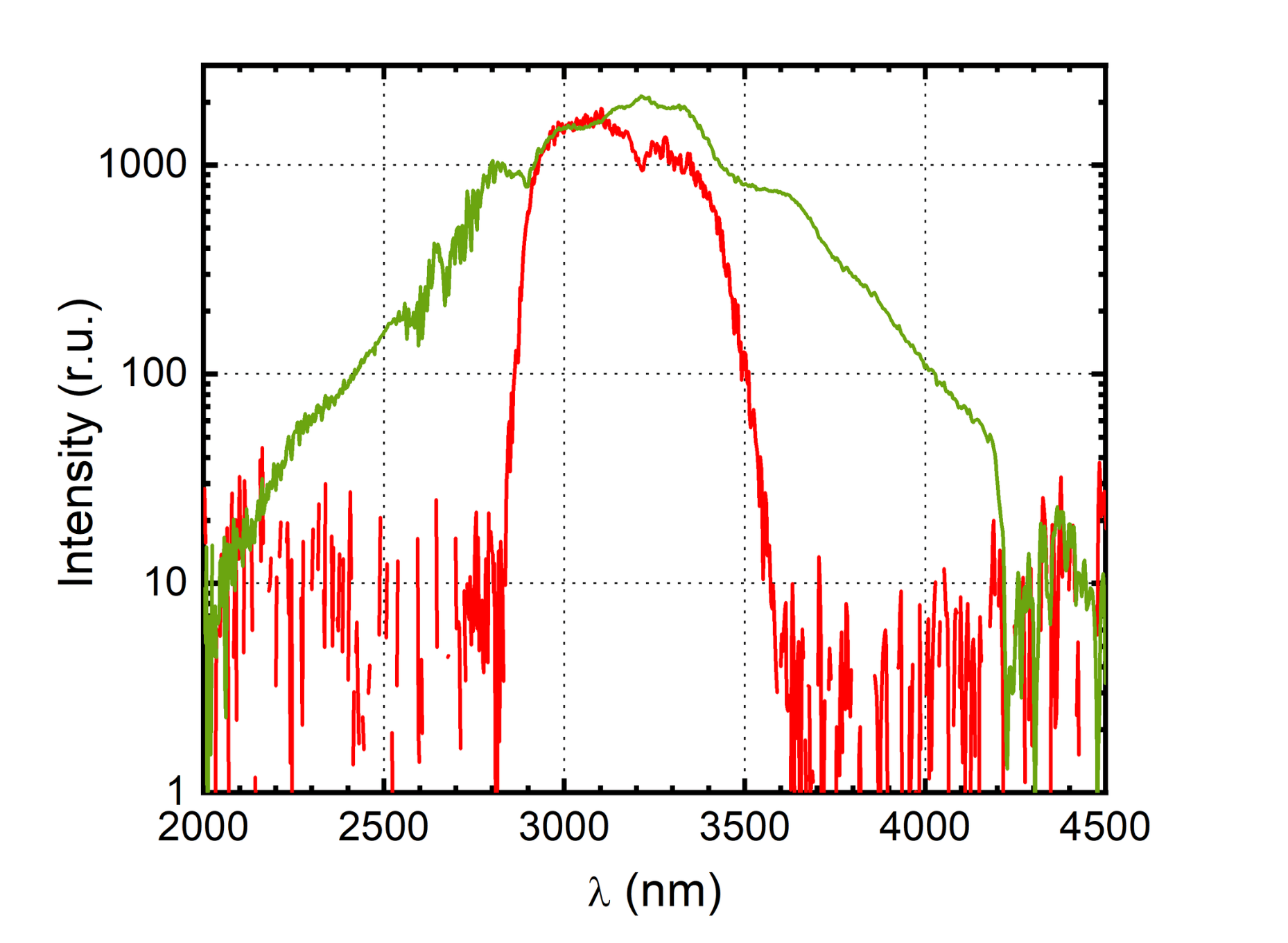
Recompression to 19 fs FWHM (1.9 cycles @ 3 μm) was successfully achieved by inserting 5 mm CaF2 together with 3 reflections on the dispersive mirrors with angle of incidences of 10-25-25 degrees. Fig. 4 shows FROG traces and retrieved time and spectrum intensities of the recompressed pulse.
Fig. 4. Measured (a) and reconstructed (b) SH-FROG traces; reconstructed temporal
intensity profile (c) and spectrum (solid line) with spectral phase (dashed line) (d).
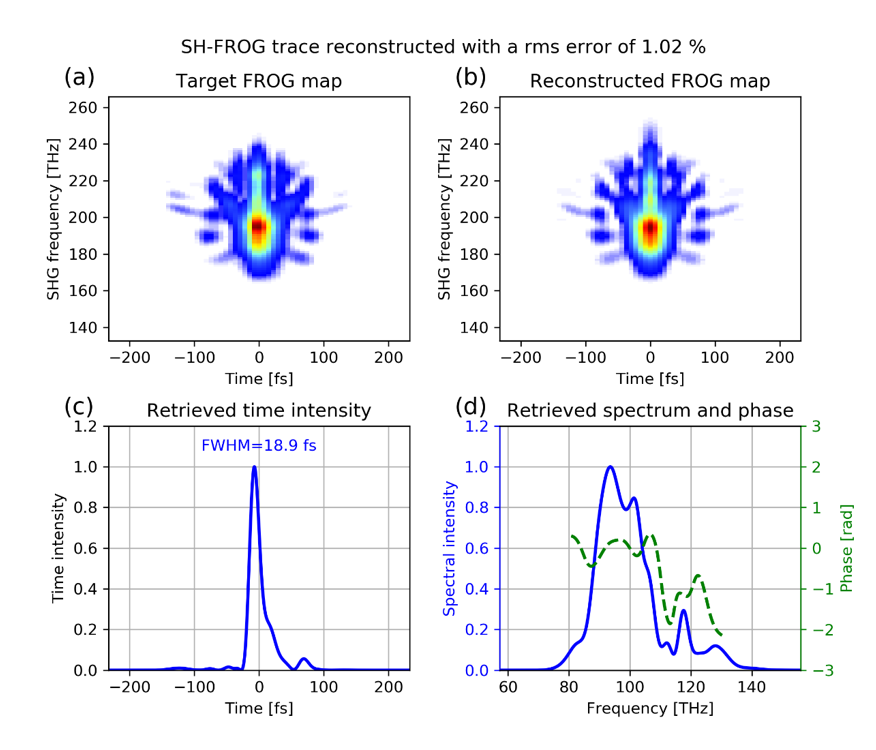
About the company
Acknowledgments
Dr. Dominik Hoff, Single Cycle Instruments